
생물기원 이차유기에어로졸의 점성도와 상 규명에 관한 최근 연구 동향
Copyright © 2016 Korean Society for Atmospheric Environment
Abstract
Researchers have traditionally assumed that aerosol particles containing secondary organic aerosols (SOAs) are to be in liquid state with low viscosity even at low relative humidity. However, recent measurements showed that SOAs can have high viscosity under certain conditions. Herein, new different techniques for measurements of viscosities of SOA particles are introduced. Moreover, laboratory studies for the viscosities and the phases of biogenic SOAs produced by α-pinene, isoprene, limonene, and β-caryophyllene of atmospheric relevance are reviewed. Future studies for determination of the phases of atmospheric aerosol particles are also suggested.
Keywords:
Viscosity, Phase, Biogenic secondary organic aerosol1. 서 론
식생, 대도시로부터 방출되는 휘발성유기화합물 (volatile organic compounds) (예: isoprene, α-pinene, toluene 등)은 대기 중 OH 라디칼, NO3 라디칼, O3와 같은 산화제와의 광화학 반응에 의해 이차유기에어로졸 (secondary organic aerosol, SOA)을 생성한다 (Hallquist et al., 2009). 이렇게 생성된 이차유기에어로졸은 지역에 따라 전체 미세에어로졸 질량의 20~80%를 차지한다 (Jimenez et al., 2009; Zhang et al., 2007). 이차유기에어로졸은 대기 중에서 직접적으로는 태양 복사를 산란 혹은 흡수하며, 간접적으로는 구름의 성질을 변화시킨다 (IPCC, 2013). 또한 시정장애 및 호흡기 질환 등과 같은 질병을 일으키는 것으로 보고된 바 있다 (Baltensperger et al., 2008; Jang et al., 2006).
최근 에어로졸의 상 (phase)을 (i.e. 액체, 반고체, 고체) 규명하기 위한 연구들이 많이 진행되고 있다. 이차유기에어로졸의 물리적 상태 (physical state)는 에어로졸 수치 모델에서 주요 인자이며, 모델 결과에 지배적인 영향을 미친다. 예를 들어, 최근 Shiraiwa and Seinfeld (2012) 모델 연구결과에서 에어로졸의 물리적 상태를 액체 상태만으로 가정하였을 경우 에어로졸을 (반)고체 상태로 계산했을 때보다 에어로졸의 질량 농도는 약 10배 정도 높게 (overprediction) 계산되었다. 또한 Shiraiwa et al. (2011) 모델 결과에서 유기에어로졸이 (반)고체 상태로 존재할 경우 유기에어로졸과 가스상 물질과의 반응 메커니즘은 흡수 (absorption)에서 흡착 (adsorption)으로 전환되며, 이차유기에어로졸과 오존과 같은 산화제와의 반응 속도는 상대습도가 90%에서 30%로 변함에 따라 약 102에서 105배까지 감소하는 것으로 나타났다. 또한 Riipinen et al. (2011)의 모델 결과에서는 초미세먼지의 수농도와 크기 분포는 이차유기에어로졸 입자의 확산속도와 점성도에 의존하는 것으로 나타났다.
이차유기에어로졸 입자의 상 (phase), 점성도 (viscosity), 확산속도 (molecular diffusion rate)는 서로 긴밀하게 연관된다. 어떤 물질의 물리적 상태는 점성도에 따라 1012 Pa s 이상일 때 고체, 102~1012 Pa s일 때 반고체, 102 Pa s 이하일 때 액체로 정의된다 (Koop et al., 2011). 예를 들어 상온에서 물의 점성도는 약 10-3 Pa s, 꿀의 경우는 점성도가 약 10 Pa s로 두 물질 모두 액체로 정의된다. 케첩, 땅콩 잼, 피치 (pitch)의 점성도는 각각 ~102 Pa s, ~103 Pa s, ~108 Pa s로 모두 반고체에 해당된다. 반면 유리의 점성도는 ~1012 Pa s로 고체로 정의된다. 물질의 점성도가 증가하면 물질의 확산속도는 감소하게 된다. 이차유기에어로졸 입자 안에서 가스상 유기물질의 확산속도는 점성도와 Stokes-Einstein 방정식을 이용하여 규명할 수 있다 (Koop et al., 2011).
그동안 이차유기에어로졸은 복잡한 화학조성 때문에 대기 중에서 액체 상태로 존재할 것으로 여겨져 왔다 (Marcolli et al., 2004). 그리고 대부분의 에어로졸 성장 모델에서 에어로졸은 점성도가 낮다고 가정되어 왔다 (Hallquist et al., 2009). 즉 에어로졸은 점성도가 낮기 때문에 에어로졸 입자 안으로 가스상 물질의 확산이 빠르게 일어나고, 에어로졸과 가스상 물질은 대기 중에서 평형상태를 이룰 것으로 가정되어 왔다. 그러나 Virtanen et al. (2010)은 α-pinene으로부터 생성된 이차유기에어로졸이 상대습도 0%일 때 임팩터 표면에서 rebound 되는 특성을 관찰함으로써 유기에어로졸이 특정 환경조건에서 (반)고체 상태로 존재할 수 있음을 최초로 발견하였다. 이를 좀 더 정성·정량적으로 명확히 파악하기 위해 최근 이차유기에어로졸의 점성도 측정, 확산속도 측정, bounce 특성 측정, 증발속도 측정 등의 새로운 기법을 이용한 시도들이 활발히 진행되고 있다.
여기서는 최신의 에어로졸 정성·정량 점성도 측정기법들을 살펴보고, 이를 이용한 생물기원 이차유기에어로졸의 상대습도에 따른 물리적 상태를 파악하고자 하였다. 특히 최근 개발된 에어로졸 점성도 직접 측정법인 bead-mobility 기법과 poke-flow 기법, 에어로졸 rebound 측정법을 소개하고, 그 기법들을 이용한 α-pinene, isoprene, limonene, β-caryophyllene으로부터 생성된 이차유기에어로졸의 점성도와 상 규명 최근 연구 동향을 살펴보는 것에 초점을 맞추었다. 또한 이러한 연구결과를 바탕으로 앞으로의 대기 에어로졸 상 규명의 연구방향을 제안하였다.
2. 이차유기에어로졸의 점성도 측정 기법
최근 세계적으로 생물기원 이차유기에어로졸의 점성도를 측정하기 위해 많은 방법들이 시도되고 있다. 표 1은 생물기원 이차유기에어로졸의 점성도 규명을 위한 다양한 기법들을 보여준다. 직접적으로 에어로졸의 점성도를 분석할 수 있는 기법은 (1) particle rebound 방법 (Bateman et al., 2015; Li et al., 2015), (2) bead-mobility와 poke-flow 방법 (Grayson et al., 2016; Hinks et al., 2016; Song et al., 2016; Grayson et al., 2015; Song et al., 2015; Renbaum-Wolff et al., 2013a, b)이 있다. 간접적으로 에어로졸의 점성도를 추정하는 기법은 (1) 에어로졸 입자 안에서의 확산속도 (diffusion rate)와 혼합시간 (mixing time)을 측정 (Abramson et al., 2013; Loza et al., 2013; Perraud et al., 2012), (2) bounce 특성 이용 (Kidd et al., 2014; Saukko et al., 2012; Virtanen et al., 2010, 2011), (3) 이차유기에어로졸의 증발률 (evaporation rate) 이용 (Cappa and Wilson, 2011; Vaden et al., 2011), (4) 이차유기에어로졸의 반응성 (reactivity) 측정 (Wang et al., 2015; Kuwata and Martin, 2012) 등이 있다. 이러한 다양한 기법들 중에서 점성도 정량 분석이 가능한 분석법을 다음 장에서 소개하겠다.

Summary of literature for the different analytical methods of biogenic secondary organic aerosols (SOAs).
2. 1 Particle rebound
에어로졸은 액체로 존재하느냐 (반)고체로 존재하느냐에 따라 임팩터에 포집되는 효율이 달라질 수 있다. 다시 말해 액체상의 에어로졸은 임팩터 plate 위에서 효율적으로 포집될 수 있지만, (반)고체상의 에어로졸은 임팩터 plate 위에서 튕겨져 나가 (rebound) 포집 효율이 현저히 줄어들게 된다. Virtanen et al. (2010)은 이러한 에어로졸의 상 (phase) 특성에 착안하여 건조 조건에서 직경 20~100 nm의 이차유기에어로졸의 물리적 상태를 정성적으로 발견하였다. 이후 Bateman et al. (2015)은 190, 240 nm의 sucrose/H2O 에어로졸의 알려진 상대습도별 점성도와 rebound fraction과의 연관성을 측정하여 semisolid-to-liquid 상 전이 (phase transition) 구간을 검증하였다. 이러한 particle rebound 특성을 측정하여 Li et al. (2015) 또한 다양한 이차유기에어로졸이 (직경 ~300 nm) 어느 상대습도에서 semisolid-to-liquid 상 전이를 경험하는지 측정하고, 액체에서 반고체로 상 전이가 일어날 때의 에어로졸 점성도를 100~102 Pa s으로 정의하였다 (표 1).
2. 2 Bead-mobility technique
미세한 크기의 에어로졸의 점성도를 측정하는 것은 기술적으로 매우 어렵다. 이러한 한계를 극복하여 University of British Columbia의 Bertram 그룹에서는 마이크로미터 크기의 에어로졸의 점성도를 측정하는 분석기법을 개발하였다 (일명: bead-mobility). Bead-mobility 방법은 Renbaum-Wolff et al. (2013b)이 소개하고 검증도 하였으며, 점성도 10-3~103 Pa s의 범위에서 측정이 가능하다. 이 방법을 간단히 소개하자면, 소수성 표면 (hydrophobic substrate) 위에 포집된 슈퍼 마이크로미터 크기의 에어로졸 입자 안에 직경 ~1 μm의 비드 (beads)를 분사시킨다. 그리고 이것을 온습도 조절용 챔버 안에 장착시킨다. 이후 일정한 상대습도에서 일정량의 초순수 질소가스를 계속 주입하면 에어로졸 입자 표면에 전단응력 (shear stress)이 발생하게 되어 에어로졸 입자 안의 비드는 일정한 속도로 움직이게 된다. 이때 일정한 시간 동안 비드의 속도를 광학현미경을 통해 관찰하고, 그 속도를 측정한다. 그림 1은 Song et al. (2015) 연구에서 isoprene으로부터 생성된 이차유기에어로졸 입자 안에서 상대습도 80%일 때의 비드의 움직임을 보여준다. 측정된 비드 평균속도는 다양한 유기물질로부터 구해낸 ‘bead 속도-점성도’ 검정선을 이용하여 점성도로 변환한다 (그림 2) (Song et al., 2015).
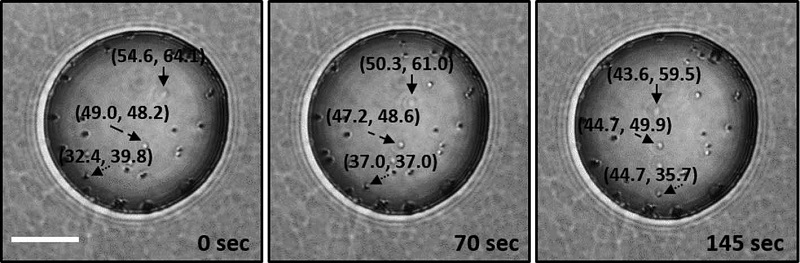
Optical images of a SOA particle produced from isoprene photo-oxidation at 80% relative humidity at 295 K which were recorded for a bead-mobility experiment. Three beads including the x and y coordinates were labeled with different arrows. Scale bar: 20 μm. The figure was taken from Song et al. (2015).
2. 3 Poke-flow technique in conjunction with fluid simulation
물리적인 힘 (physical force)을 이용하여 에어로졸 입자의 상 (phase)을 추정하는 방법은 Murray et al. (2012)에 의해 처음 소개되었다. 이후 Renbaum-Wolff et al. (2013a)은 일명 poke-flow 측정법과 유체흐름 시뮬레이션 (fluid simulation)을 접목하여 에어로졸의 점성도를 정량적으로 규명할 수 있도록 확장하였고, Grayson et al. (2015)이 그 방법을 검증하였다. Poke flow-유체시뮬레이션 방법은 bead-mobility 방법보다 높은 점성도인 103~1012 Pa s 범위에서 분석이 가능하다. 이 방법을 간단히 소개하면, 소수성 표면 위에 포집된 슈퍼 마이크로미터 크기의 에어로졸을 온습도 조절용 챔버 안에 장착시킨다. 챔버 윗면에 바늘 (needle)을 삽입할 수 있는 작은 윈도우 (window)가 있다. 일정한 상대습도에서 에어로졸을 바늘로 포킹 (poking)하면 에어로졸은 half-torus geometry를 형성하게 된다. 이때 유체는 표면에너지 (surface energy)를 최소화하기 위해 유체의 안쪽으로 흐르게 되며, 에어로졸이 원래의 형태로 복원(recovery)되는 시간을 측정한다. 그림 3은 poke-flow 방법을 이용한 isoprene으로부터 생성된 이차유기에어로졸의 포킹 전과 후의 형태 변화를 보여준다 (Song et al., 2015).
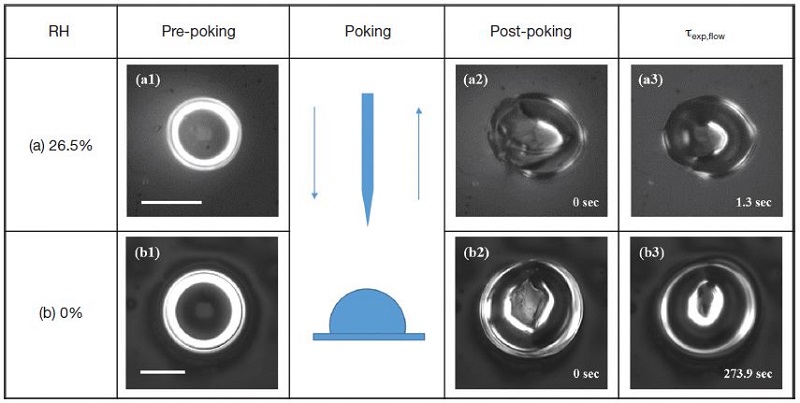
Optical images of SOA particles produced from isoprene photo-oxidation poked at (a) 26.5% RH and (b) 0% RH at 295 K during typical poke-flow experiments. Images (a1) and (b1) correspond to the particles pre-poking. Images (a2) and (b2) correspond to the first frame post-poking (time set=0 s). Images (a3) and (b3) correspond to images of the experimental flow time, τexp, when the diameter of the hole has decreased to 50% of its original size. Scale bar: 20 μm. The figure was taken from Song et al. (2015).
Poke-flow 실험 이후 유체흐름 시뮬레이션 (COMSOL Multiphysics)을 수행하였다. 시뮬레이션 방법은 Renbaum-Wolff et al. (2013a)과 Grayson et al. (2015)에 자세히 서술되어 있다. 유체흐름 시뮬레이션으로부터 유체흐름 시간과 점성도의 상관성을 계산하였고, 이때의 상관성을 이용하여 poke-flow 실험에서 측정한 에어로졸의 recovery time을 에어로졸의 점성도로 변환하였다.
3. 생물기원 이차유기에어로졸의 점성도와 상
현재까지 대기 에어로졸의 물리적인 상태를 in-situ로 관측할 수 있는 기술이 없으므로 실험실 기반의 연구가 필요하다. 따라서 실험실 기반에서 이차유기에어로졸을 생성하여 그 점성도를 측정 혹은 유추하고, 이차유기에어로졸의 상을 예측하는 연구들이 수행되고 있다. 숲, 공장지역, 도시대기 등 다양한 환경으로부터 배출되는 α-pinene, isoprene, limonene 등과 같은 휘발성유기화합물은 전 지구적 규모에서 전체 휘발성유기화합물 배출량의 약 90%까지 차지하고 있으며, 연간 900억 kg의 이차유기에어로졸을 생성하는 것으로 보고된 바 있다 (Hallquist et al., 2009; Kanakidou et al., 2005). 따라서 생물기원 이차유기에어로졸은 에어로졸 물리적 상태 규명 연구에서 대기에어로졸을 대표하는 물질로 활용되고 있다.
3. 1 α-pinene으로부터 생성된 이차유기에어로졸
생물기원 이차유기에어로졸 중에서 α-pinene으로부터 생성된 이차유기에어로졸은 가장 활발히 연구되었다. 표 1은 이차유기에어로졸의 점성도 선행연구들의 결과 값을 보여준다. 이차유기에어로졸은 α-pinene과 O3 (ozonolysis 메커니즘) 또는 OH (photo-oxidation 메커니즘)가 관여되는 두 가지 반응에 의해 생성되었다 (표 1). Virtanen et al. (2010)은 α-pinene photo-oxidation, α-pinene ozonolysis로부터 생성된 이차유기에어로졸을 이용하여 건조 조건에서 에어로졸이 rebound 되는 특성을 관찰하고, 이로부터 건조 조건에서 이차유기에어로졸은 기존의 가설 (유기에어로졸은 점성도가 낮다)보다 상당히 높은 점성도를 갖는다고 제시하였다. 이후 Saukko et al. (2012)과 Pajunoja et al. (2015)의 연구에서도 α-pinene photo-oxidation으로부터 생성된 이차유기에어로졸이 rebound 되는 특성을 측정하여 이 에어로졸의 높은 점성도를 유추하였다. 그러나 이러한 α-pinene photo-oxidation으로부터 생성된 이차유기에어로졸의 점성도 연구는 정성적인 결과로 정량적인 점성도의 값은 제시되지 못하였다.
α-pinene ozonolysis로부터 생성된 이차유기에어로졸의 상태 연구는 α-pinene photo-oxidation 연구보다 좀 더 활발히 진행되었다 (표 1). 에어로졸의 rebound 특성을 이용하여 특정 환경에서 높은 점성도가 추정되었다 (Bateman et al., 2015; Kidd et al., 2014; Pajunoja et al., 2014; Virtanen et al., 2010). 또한 건조 조건에서 에어로졸 입자의 확산 계수를 측정하여 점성도를 계산하였다 (Abramson et al., 2013; Perraud et al., 2012). Grayson et al. (2016)의 연구에서는 이차유기에어로졸의 생성 조건을 변화시켜 α-pinene 이차유기에어로졸을 준비하고, poke-flow technique을 이용하여 상대습도 50~0% 범위에서 점성도를 측정하였다. 그림 4a는 α-pinene으로부터 생성된 이차유기에어로졸 점성도 선 행연구 결과를 보여준다. 선행연구에서 다양한 기법을 이용하여 α-pinene 이차유기에어로졸의 점성도를 측정하였다 (표 1 참고). 각각의 기법에 따라 약간의 차이를 보이고 있지만, 전체적으로 상대습도 70% 이상에서 α-pinene 이차유기에어로졸의 점성도는 ~102 Pa s 이하였으며, 상대습도 70%에서 0%로 감소함에 따라 점성도는 ~102 Pa s에서 ~1012 Pa s로 증가하였다. 즉, 상대습도 70% 이상에서 α-pinene 이차유기에어로졸은 액체 상태이며, 상대습도 70% 이하에서 반고체 상태로 존재하였다. Renbaum-Wolff et al. (2013a)은 수용성 (water-soluble) α-pinene 이차유기에어로졸의 점성도를 bead-mobility와 poke-flow 방법으로 측정하였다 (하늘색, 그림 4a). 수용성 α-pinene 이차유기에어로졸은 total α-pinene 이차유기에어로졸의 점성도보다 현저히 높은 점성도를 나타냈다. 상대습도 85% 이상에서 수용성 α-pinene 이차유기에어로졸의 점성도는 ~102 Pa s 이하로, 상대습도 75%에서 40%로 감소함에 따라 점성도는 약 102 Pa s에서 107 Pa s로 증가하였으며, 상대습도 30% 미만에서 ~109 Pa s 이상이었다. 이로부터 수용성 α-pinene 이차유기에어로졸은 상대습도 85% 이상에서 액체 상태, 40~75% 상대습도에서 반고체 상태, 30% 미만에서 반고체 혹은 고체 상태로 존재하는 것으로 나타났다.
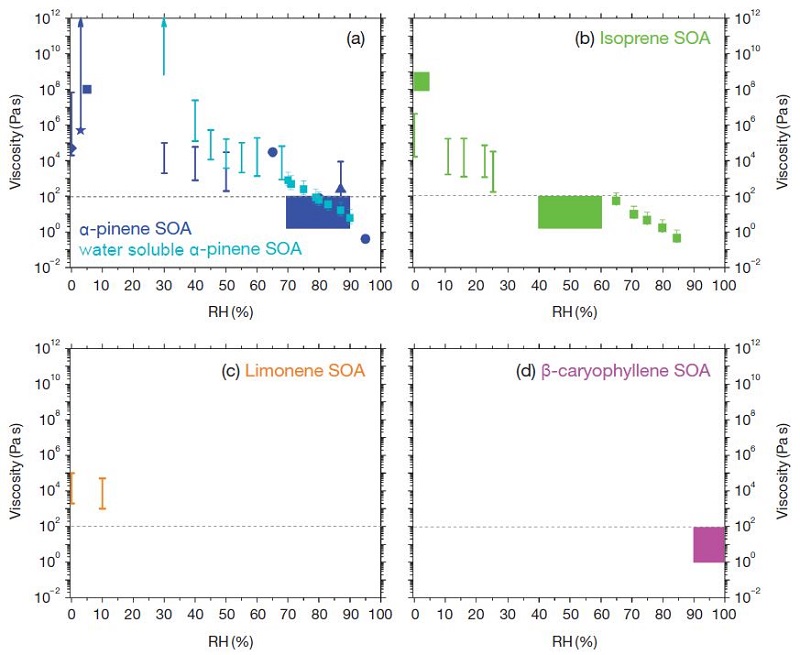
Viscosities as a function of RH of biogenic secondary organic aerosol (SOA) particles at 290 K~300 K. (a) Viscosities of α-pinene-derived SOA from literature (Perraud et al., 2012, star; Abramson et al., 2013, square; Robinson et al., 2013, diamond; Bateman et al., 2014, box; Kidd et al., 2014, triangle; and Wang et al., 2014, circle) and viscosities of water-soluble α-pinene-derived SOA particles from Renbaum-Wolff et al. (2013a) (cyan). (b) Viscosities of isoprene-derived SOA from literature (Bateman et al., 2015, big box; Li et al., 2015, small box; Song et al., 2015, error bar). (c) Viscosities of limonene-derived SOA (Hinks et al., 2016). (d) Viscosities of β-caryophyllene-derived SOA (Li et al., 2015) (see also Table 1).
3. 2 Isoprene으로부터 생성된 이차유기에어로졸
그림 4b는 isoprene으로부터 생성된 이차유기에어로졸의 상대습도별 점성도 결과를 보여준다 (표 1 참고). Bateman et al. (2015)은 isoprene photo-oxidation으로부터 생성된 이차유기에어로졸이 고체 표면에서 rebound 되는 특성을 이용하여 상대습도 60~40%에서 점성도가 100 Pa s에서 102 Pa s으로 나타나는 것을 보고하였다. 이후 Song et al. (2015)은 bead-mobility와 poke-flow 방법을 이용하여 isoprene photo-oxidation으로부터 생성된 이차유기에어로졸의 점성도를 정량적으로 측정하였다. Isoprene 이차유기에어로졸의 점성도는 상대습도 40% 이상에서 ~102 Pa s 이하인 것으로 나타났으며, 상대습도 25%에서 0%로 감소함에 따라 점성도는 ~102 Pa s에서 ~5×105 Pa s로 증가하였다. Bateman et al. (2015)과 Song et al. (2015)의 다른 기법을 이용한 점성도 연구결과는 상대습도 40~60% 범위에서 잘 일치하였다. 또한 Li et al. (2015)는 건조 조건에서 isoprene 이차유기에어로졸과 NH3의 반응속도로부터 isoprene SOA의 점성도를 약 108~109 Pa s로 정의하였다. 이 결과는 건조 조건에서 ~5×105 Pa s로 나타난 Song et al. (2015)의 결과보다는 상당히 높게 나타났다. 종합해 보면 isoprene photo-oxidation으로부터 생성된 이차유기에어로졸은 상대습도 40% 이상에서는 액체, 25% 미만에서는 반고체로 존재하는 것으로 나타났다.
3. 3 Limonene으로부터 생성된 이차유기에어로졸
Hinks et al. (2016)은 limonene ozonolysis로부터 생성된 이차유기에어로졸의 점성도를 측정하였다. 그림 4c는 낮은 상대습도에서 측정된 limonene 이차유기에어로졸의 점성도를 나타낸다. 상대습도 10%와 0%에서 조사되었으며 각각 ~104±1 Pa s, 2×104±1 Pa s 값을 보였다. Hinks et al. (2016) 연구결과로부터 낮은 상대습도에서 limonene 이차유기에어로졸은 반고체 상태인 것으로 판단된다.
3. 4 β-caryophyllene으로부터 생성된 이차유기에어로졸
현재까지 β-caryophyllene으로부터 생성된 이차유기에어로졸의 물리적 상태 연구는 Li et al. (2015)이 유일하다. Li et al. (2015)은 β-caryophyllene photo-oxidation으로부터 생성된 이차유기에어로졸로 에어로졸 rebound 특성 연구를 수행하였다. 그림 4d는 그 결과를 보여준다. 상대습도 90% 이상에서 β-caryophyllene 이차유기에어로졸은 점성도 100~102 Pa s인 것으로 조사되었다. 이는 앞에서 언급된 다른 종류의 이차유기에어로졸들의 점성도 값보다 현저히 높은 것으로 나타났다. β-caryophyllene 이차유기에어로졸은 상대습도 90% 에서 이미 반고체 상태 혹은 고체 상태일 것으로 판단되었다.
Song et al. (2016)은 세계 15개 대도시들 (도쿄, 델리, 상하이, 멕시코시티, 상파울로, 뭄바이, 오사카, 베이징, 뉴욕, 카이로, 다카, 카라치, 부에노스아이레스, 콜카타, 이스탄불)의 2004~2014년 기간 동안 월평균 상대습도를 조사하였다. 이러한 대도시 지역은 인위적으로 생성된 이차유기에어로졸뿐만 아니라 생물기원의 이차유기에어로졸의 생성도 빈번한 것으로 보고되고 있다 (Jimenez et al., 2009). 상대습도는 지역과 시간에 따라 변화를 보였고, 월평균 상대습도는 10~90% 범위로 조사되었다. 이러한 도시들의 상대습도 분포와 생물기원 이차유기에어로졸 점성도 연구결과를 감안할 때, 대기 중에서 생물기원의 이차유기에어로졸은 액체뿐만 아니라 반고체 혹은 고체로도 존재할 수 있을 것으로 예상되었다.
4. 요 약
- (1) 전 세계적으로 최근 다양한 분석방법을 개발하여 이차유기에어로졸의 물리적 상태 (physical state)를 추정하고 있다. 정성적인 분석법으로는 이차유기에어로졸의 확산속도 (diffusion rate) 측정, bounce 특성 측정, 증발속도 측정, 가스상 물질과의 반응성 측정법이 있다. 정량적인 분석법으로는 bead-mobility, poke-flow 방법, rebound 특성 측정법이 있다.
- (2) 다양한 종류의 생물기원 이차유기에어로졸의 점성도가 조사되었다. α-pinene으로부터 생성된 이차유기에어로졸은 상대습도 70% 이상에서 ~102 Pa s 이하, 70~0%에서 약 102 Pa s~1012 Pa s였고, 수용성 α-pinene 이차유기에어로졸은 상대습도 85% 이상에서 ~102 Pa s 이하, 75~40%에서 ~102 Pa s~107 Pa s, 30% 미만에서 ~109 Pa s 이상인 것으로 나타났다. 또한 isoprene으로부터 생성된 이차유기에어로졸은 상대습도 40% 이상에서 ~102 Pa s 이하, 25~0% 상대습도에서 약 102 Pa s~109 Pa s로 조사되었다. Limonene으로부터 생성된 이차유기에어로졸은 상대습도 10% 미만에서 ~103 Pa s 이상으로 측정되었다. β-caryophyllene 이차유기에어로졸은 상대습도 90% 이상에서 점성도 100 Pa s~102 Pa s인 것으로 추정되었다.
- (3) 다양한 종류의 생물기원 이차유기에어로졸의 상대습도별 점성도는 limonene 이차유기에어로졸<isoprene 이차유기에어로졸<α-pinene 이차유기에어로졸<β-caryophyllene 이차유기에어로졸의 순으로 나타났다. 또한 α-pinene 이차유기에어로졸과 수용성 α-pinene 이차유기에어로졸의 점성도 비교 결과 수용성 이차유기에어로졸의 점성도가 같은 상대습도에서 현저히 높게 나타났다.
- (4) 물질의 물리적 상태는 점성도에 따라 1012 Pa s 이상일 때 고체, 102~1012 Pa s일 때 반고체, 102 Pa s 이하일 때 액체로 정의된다. 지금까지의 연구결과로부터 α-pinene으로부터 생성된 이차유기에어로졸은 상대습도 70% 이상에서 액체, 70% 미만에서 반고체로 존재하였다. 그리고 수용성 α-pinene 이차유기에어로졸의 경우에는 상대습도 85% 이상에서 액체, 75~40%에서 반고체, 30% 미만에서 반고체 혹은 고체 상태인 것으로 나타났다. Isoprene으로부터 생성된 이차유기에어로졸은 상대습도 40% 이상에서 액체, 40% 미만에서 반고체로 존재하였다. 또한 limonene으로부터 생성된 이차유기에어로졸은 낮은 상대습도에서 반고체로 존재함을 보여주었다. β-caryophyllene 이차유기에어로졸의 경우에는 높은 상대습도 (90% 미만)에서도 이미 반고체 상태로 존재하는 것으로 조사되었다. 이러한 선행연구로부터 생물기원 이차유기에어로졸은 대기환경 조건에서 액체뿐만 아니라 반고체 혹은 고체 상태로 존재할 수 있음이 제시되었다.
5. 전 망
최근 5년간 생물기원 이차유기에어로졸의 물리적 상태를 규명하기 위한 연구가 많이 진행되었지만, 앞으로도 더 많은 연구가 수행되어야 한다. 지금까지의 연구결과를 기반으로 다음과 같은 앞으로 수행되어야 할 연구 방향을 제시한다.
- (1) 인위적 기원의 이차유기에어로졸 연구. 대도시와 같은 지역에서는 인위적 기원의 이차유기에어로졸 농도가 현저하다. 그러나 아직까지 인위적 기원의 이차유기에어로졸의 상 연구는 매우 한정적이다. 이러한 이차유기에어로졸도 액체 상태뿐만 아니라 반고체, 고체로 존재하는지에 관한 더 많은 연구가 필요하다.
- (2) 유기성분과 무기염이 혼합된 에어로졸 연구. 대기 중에서 에어로졸은 유기성분과 무기염이 혼합된 형태로 존재한다고 보고된 바 있다. 따라서 무기염이 첨가된 에어로졸 입자 안에서 습도 변화에 의해 점성도가 어떻게 달라지는지에 관한 연구가 수행되어야 한다.
- (3) 온도 변화 연구. 현재까지 대부분의 연구는 290~ 300 K 온도에서 수행되었다. 점성도는 온도에 의존되며, 온도가 낮아짐에 따라 에어로졸은 더 큰 점성도를 갖게 될 것으로 예상된다. 특히 낮은 온도에서의 에어로졸 점성도 연구는 상층 대기에서 에어로졸의 역할을 이해하는 데 필수적이다. 그러므로 온도에 따라 에어로졸의 물리적 상태가 어떻게 변하는지에 관한 연구가 요구된다.
- (4) 대기 에어로졸 연구. 지금까지 실제 대기 에어로졸의 물리적 상태는 보고된 바 없다. 실험실 환경에서 규명한 에어로졸의 점성도와 물리적 상태에 관한 연구결과가 대기 에어로졸에서도 일치하는지 검증하는 작업이 필요하다. 이를 위해 다양한 지역에서 대기 에어로졸을 포집하여 성분분석과 함께 에어로졸의 상을 규명하는 연구가 수행되어야 한다.
제안된 연구를 통해 지금까지 난제로 남아 있는 에어로졸의 기본적인 물리·화학적 특성을 밝혀냄으로써 좀 더 정확한 에어로졸의 역할과 영향을 이해할 수 있을 것이다. 에어로졸이 대기 중에서 액체상으로 존재하는지 (반)고체 상으로 존재하는지에 관해 규명함으로써 현재까지 예측이 불분명한 에어로졸 크기 분포, 성장 속도, 산출량, 수송 패턴 등에 관한 모델링 연구에 적극 활용될 것으로 전망된다 (서론 참고). 이러한 연구는 대기화학, 대기질, 기후변화를 좀 더 정확히 예측하는 데 기여할 것이다.
Acknowledgments
이 성과는 2016년도 정부 (미래창조과학부)의 재원으로 한국연구재단의 지원을 받아 수행된 연구입니다 (2016R1C1B1009243).
References
-
Abramson, E., D. Imre, J. Beranek, J. Wilson, and A. Zelenyuk, (2013), Experimental determination of chemical diffusion within secondary organic aerosol particles, Physical Chemistry Chemical Physics, 15, p2983-2991.
[https://doi.org/10.1039/c2cp44013j]
-
Baltensperger, U., J. Dommen, R. Alfarra, J. Duplissy, K. Gaeggeler, A. Metzger, M.C. Facchini, S. Decesari, E. Finessi, C. Reinnig, M. Schott, J. Warnke, T. Hoffmann, B. Klatzer, H. Puxbaum, M. Geiser, M. Savi, D. Lang, M. Kalberer, and T. Geiser, (2008), Combined determination of the chemical composition and of health effects of secondary organic aerosols: The POLYSOA project, Journal of Aerosol Medicine and Pulmonary Drug Delivery, 21, p145-154.
[https://doi.org/10.1089/jamp.2007.0655]
-
Bateman, A.P., A.K. Bertram, and S.T. Martin, (2015), Hygroscopic Influence on the Semisolid-to-Liquid Transition of Secondary Organic Materials, Journal of Physical Chemistry A, 119, p4386-4395.
[https://doi.org/10.1021/jp508521c]
-
Cappa, C.D., and K.R. Wilson, (2011), Evolution of organic aerosol mass spectra upon heating: implications for OA phase and partitioning behavior, Atmospheric Chemistry and Physics, 11, p1895-1911.
[https://doi.org/10.5194/acp-11-1895-2011]
-
Grayson, J.W., M. Song, M. Sellier, and A.K. Bertram, (2015), Validation of the poke-flow technique combined with simulations of fluid flow for determining viscosities in samples with small volumes and high viscosities, Atmospheric Measurement Techniques, 8, p2463-2472.
[https://doi.org/10.5194/amt-8-2463-2015]
-
Grayson, J.W., Y. Zhang, A. Mutzel, L. Renbaum-Wolff, O. Boge, S. Kamal, H. Herrmann, S.T. Martin, and A.K. Bertram, (2016), Effect of varying experimental conditions on the viscosity of alpha-pinene derived secondary organic material, Atmospheric Chemistry and Physics, 16, p6027-6040.
[https://doi.org/10.5194/acp-16-6027-2016]
-
Hallquist, M., J.C. Wenger, U. Baltensperger, Y. Rudich, D. Simpson, M. Claeys, J. Dommen, N.M. Donahue, C. George, A.H. Goldstein, J.F. Hamilton, H. Herrmann, T. Hoffmann, Y. Iinuma, M. Jang, M.E. Jenkin, J.L. Jimenez, A. Kiendler-Scharr, W. Maenhaut, G. McFiggans, T.F. Mentel, A. Monod, A.S.H. Prevot, J.H. Seinfeld, J.D. Surratt, R. Szmigielski, and J. Wildt, (2009), The formation, properties and impact of secondary organic aerosol: current and emerging issues, Atmospheric Chemistry and Physics, 9, p5155-5236.
[https://doi.org/10.5194/acp-9-5155-2009]
-
Hinks, M.L., M.V. Brady, H. Lignell, M.J. Song, J.W. Grayson, A.K. Bertram, P. Lin, A. Laskin, J. Laskin, and S.A. Nizkorodov, (2016), Effect of viscosity on photodegradation rates in complex secondary organic aerosol materials, Physical Chemistry Chemical Physics, 18, p8785-8793.
[https://doi.org/10.1039/C5CP05226B]
- IPCC: Climate Change, (2013), The Physical Science Basis. Contribution of Working Group I to the Fifth Assessment Report of the Intergovernmental Panel on Climate Change, Edited by T. F. Stocker, D. Qin, G.-K. Plattner, M. Tignor, S. K. Allen, J. Boschung, A. Nauels, Y. Xia, V. Bex, and P. M. Midgley, Cambridge University Press, Cambridge, UK and New York, NY, USA., 1535, 2013.
-
Jang, Y., G. Kim, and D.A. Chiriboga, (2006), Correlates of sense of control among older Korean-American immigrants: Financial status, physical health constraints, and environmental challenges, International Journal of Aging and Human Development, 63, p173-186.
[https://doi.org/10.2190/9QMQ-TG4A-1LDC-CNRR]
-
Jimenez, J.L., M.R. Canagaratna, N.M. Donahue, A.S.H. Prevot, Q. Zhang, J.H. Kroll, P.F. DeCarlo, J.D. Allan, H. Coe, N.L. Ng, A.C. Aiken, K.S. Docherty, I.M. Ulbrich, A.P. Grieshop, A.L. Robinson, J. Duplissy, J.D. Smith, K.R. Wilson, V.A. Lanz, C. Hueglin, Y.L. Sun, J. Tian, A. Laaksonen, T. Raatikainen, J. Rautiainen, P. Vaattovaara, M. Ehn, M. Kulmala, J.M. Tomlinson, D.R. Collins, M.J. Cubison, E.J. Dunlea, J.A. Huffman, T.B. Onasch, M.R. Alfarra, P.I. Williams, K. Bower, Y. Kondo, J. Schneider, F. Drewnick, S. Borrmann, S. Weimer, K. Demerjian, D. Salcedo, L. Cottrell, R. Griffin, A. Takami, T. Miyoshi, S. Hatakeyama, A. Shimono, J.Y. Sun, Y.M. Zhang, K. Dzepina, J.R. Kimmel, D. Sueper, J.T. Jayne, S.C. Herndon, A.M. Trimborn, L.R. Williams, E.C. Wood, A.M. Middlebrook, C.E. Kolb, U. Baltensperger, and D.R. Worsnop, (2009), Evolution of Organic Aerosols in the Atmosphere, Science, 326, p1525-1529.
[https://doi.org/10.1126/science.1180353]
-
Kanakidou, M., J.H. Seinfeld, S.N. Pandis, I. Barnes, F.J. Dentener, M.C. Facchini, R. Van Dingenen, B. Ervens, A. Nenes, C.J. Nielsen, E. Swietlicki, J.P. Putaud, Y. Balkanski, S. Fuzzi, J. Horth, G.K. Moortgat, R. Winterhalter, C.E.L. Myhre, K. Tsigaridis, E. Vignati, E.G. Stephanou, and J. Wilson, (2005), Organic aerosol and global climate modelling: a review, Atmospheric Chemistry and Physics, 5, p1053-1123.
[https://doi.org/10.5194/acp-5-1053-2005]
-
Kidd, C., V. Perraud, L.M. Wingen, and B.J. Finlayson-Pitts, (2014), Integrating phase and composition of secondary organic aerosol from the ozonolysis of alpha-pinene, Proceedings of the National Academy of Sciences of the United States of America, 111, p7552-7557.
[https://doi.org/10.1073/pnas.1322558111]
-
Koop, T., J. Bookhold, M. Shiraiwa, and U. Poschl, (2011), Glass transition and phase state of organic compounds: dependency on molecular properties and implications for secondary organic aerosols in the atmosphere, Physical Chemistry Chemical Physics, 13, p19238-19255.
[https://doi.org/10.1039/c1cp22617g]
-
Kuwata, M., and S.T. Martin, (2012), Phase of atmospheric secondary organic material affects its reactivity, Proceedings of the National Academy of Sciences of the United States of America, 109, p17354-17359.
[https://doi.org/10.1073/pnas.1209071109]
-
Li, Y.J., P.F. Liu, Z.H. Gong, Y. Wang, A.P. Bateman, C. Bergoend, A.K. Bertram, and S.T. Martin, (2015), Chemical Reactivity and Liquid/Nonliquid States of Secondary Organic Material, Environmental Science and Technology, 49, p13264-13274.
[https://doi.org/10.1021/acs.est.5b03392]
-
Loza, C.L., M.M. Coggon, T.B. Nguyen, A. Zuend, R.C. Flagan, and J.H. Seinfeld, (2013), On the Mixing and Evaporation of Secondary Organic Aerosol Components, Environmental Science and Technology, 47, p6173-6180.
[https://doi.org/10.1021/es400979k]
-
Marcolli, C., B.P. Luo, and T. Peter, (2004), Mixing of the organic aerosol fractions: Liquids as the thermodynamically stable phases, Journal of Physical Chemistry A, 108, p2216-2224.
[https://doi.org/10.1021/jp036080l]
-
Murray, B.J., A.E. Haddrell, S. Peppe, J.F. Davies, J.P. Reid, D. O’Sullivan, H.C. Price, R. Kumar, R.W. Saunders, J.M.C. Plane, N.S. Umo, and T.W. Wilson, (2012), Glass formation and unusual hygroscopic growth of iodic acid solution droplets with relevance for iodine mediated particle formation in the marine boundary layer, Atmospheric Chemistry and Physics, 12, p8575-8587.
[https://doi.org/10.5194/acp-12-8575-2012]
-
Pajunoja, A., A.T. Lambe, J. Hakala, N. Rastak, M.J. Cummings, J.F. Brogan, L.Q. Hao, M. Paramonov, J. Hong, N.L. Prisle, J. Malila, S. Romakkaniemi, K.E.J. Lehtinen, A. Laaksonen, M. Kulmala, P. Massoli, T.B. Onasch, N.M. Donahue, I. Riipinen, P. Davidovits, D.R. Worsnop, T. Petaja, and A. Virtanen, (2015), Adsorptive uptake of water by semisolid secondary organic aerosols, Geophysical Research Letters, 42, p3063-3068.
[https://doi.org/10.1002/2015GL063142]
-
Pajunoja, A., J. Malila, L.Q. Hao, J. Joutsensaari, K.E.J. Lehtinen, and A. Virtanen, (2014), Estimating the Viscosity Range of SOA Particles Based on Their Coalescence Time, Aerosol Science and Technology, 48, pI-Iv.
[https://doi.org/10.1080/02786826.2013.870325]
-
Perraud, V., E.A. Bruns, M.J. Ezell, S.N. Johnson, Y. Yu, M.L. Alexander, A. Zelenyuk, D. Imre, W.L. Chang, D. Dabdub, J.F. Pankow, and B.J. Finlayson-Pitts, (2012), Nonequilibrium atmospheric secondary organic aerosol formation and growth, Proceedings of the National Academy of Sciences of the United States of America, 109, p2836-2841.
[https://doi.org/10.1073/pnas.1119909109]
-
Renbaum-Wolff, L., J.W. Grayson, A.P. Bateman, M. Kuwata, M. Sellier, B.J. Murray, J.E. Shilling, S.T. Martin, and A.K. Bertram, (2013a), Viscosity of alpha-pinene secondary organic material and implications for particle growth and reactivity, Proceedings of the National Academy of Sciences of the United States of America, 110, p8014-8019.
[https://doi.org/10.1073/pnas.1219548110]
-
Renbaum-Wolff, L., J.W. Grayson, and A.K. Bertram, (2013b), Technical Note: New methodology for measuring viscosities in small volumes characteristic of environmental chamber particle samples, Atmospheric Chemistry and Physics, 13, p791-802.
[https://doi.org/10.5194/acp-13-791-2013]
-
Riipinen, I., J.R. Pierce, T. Yli-Juuti, T. Nieminen, S. Hakkinen, M. Ehn, H. Junninen, K. Lehtipalo, T. Petaja, J. Slowik, R. Chang, N.C. Shantz, J. Abbatt, W.R. Leaitch, V.M. Kerminen, D.R. Worsnop, S.N. Pandis, N.M. Donahue, and M. Kulmala, (2011), Organic condensation: a vital link connecting aerosol formation to cloud condensation nuclei (CCN) concentrations, Atmospheric Chemistry and Physics, 11, p3865-3878.
[https://doi.org/10.5194/acp-11-3865-2011]
-
Saukko, E., A.T. Lambe, P. Massoli, T. Koop, J.P. Wright, D.R. Croasdale, D.A. Pedernera, T.B. Onasch, A. Laaksonen, P. Davidovits, D.R. Worsnop, and A. Virtanen, (2012), Humidity-dependent phase state of SOA particles from biogenic and anthropogenic precursors, Atmospheric Chemistry and Physics, 12, p7517-7529.
[https://doi.org/10.5194/acp-12-7517-2012]
-
Shiraiwa, M., and J.H. Seinfeld, (2012), Equilibration timescale of atmospheric secondary organic aerosol partitioning, Geophysical Research Letters, 39.
[https://doi.org/10.1029/2012gl054008]
-
Shiraiwa, M., M. Ammann, T. Koop, and U. Poschl, (2011), Gas uptake and chemical aging of semisolid organic aerosol particles, Proceedings of the National Academy of Sciences of the United States of America, 108, p11003-11008.
[https://doi.org/10.1073/pnas.1103045108]
-
Song, M., P.F. Liu, S.J. Hanna, R.A. Zaveri, K. Potter, Y. You, S.T. Martin, and A.K. Bertram, (2016), Relative humidity-dependent viscosity of secondary organic material from toluene photo-oxidation and possible implications for organic particulate matter over megacities, Atmospheric Chemistry and Physics, 16, p8817-8830.
[https://doi.org/10.5194/acp-16-8817-2016]
-
Song, M., P.F. Liu, S.J. Hanna, Y.J. Li, S.T. Martin, and A.K. Bertram, (2015), Relative humidity-dependent viscosities of isoprene-derived secondary organic material and atmospheric implications for isoprenedominant forests, Atmospheric Chemistry and Physics, 15, p5145-5159.
[https://doi.org/10.5194/acp-15-5145-2015]
-
Vaden, T.D., D. Imre, J. Beranek, M. Shrivastava, and A. Zelenyuk, (2011), Evaporation kinetics and phase of laboratory and ambient secondary organic aerosol, Proceedings of the National Academy of Sciences of the United States of America, 108, p2190-2195.
[https://doi.org/10.1073/pnas.1013391108]
-
Virtanen, A., J. Joutsensaari, T. Koop, J. Kannosto, P. Yli-Pirila, J. Leskinen, J.M. Makela, J.K. Holopainen, U. Poschl, M. Kulmala, D.R. Worsnop, and A. Laaksonen, (2010), An amorphous solid state of biogenic secondary organic aerosol particles, Nature, 467, p824-827.
[https://doi.org/10.1038/nature09455]
-
Virtanen, A., J. Kannosto, H. Kuuluvainen, A. Arffman, J. Joutsensaari, E. Saukko, L. Hao, P. Yli-Pirila, P. Tiitta, J.K. Holopainen, J. Keskinen, D.R. Worsnop, J.N. Smith, and A. Laaksonen, (2011), Bounce behavior of freshly nucleated biogenic secondary organic aerosol particles, Atmospheric Chemistry and Physics, 11, p8759-8766.
[https://doi.org/10.5194/acp-11-8759-2011]
-
Wang, B.B., R.E. O’Brien, S.T. Kelly, J.E. Shilling, R.C. Moffet, M.K. Gilles, and A. Laskin, (2015), Reactivity of Liquid and Semisolid Secondary Organic Carbon with Chloride and Nitrate in Atmospheric Aerosols, Journal of Physical Chemistry A, 119, p4498-4508.
[https://doi.org/10.1021/jp510336q]
-
Zhang, Q., J.L. Jimenez, M.R. Canagaratna, J.D. Allan, H. Coe, I. Ulbrich, M.R. Alfarra, A. Takami, A.M. Middlebrook, Y.L. Sun, K. Dzepina, E. Dunlea, K. Docherty, P.F. DeCarlo, D. Salcedo, T. Onasch, J.T. Jayne, T. Miyoshi, A. Shimono, S. Hatakeyama, N. Takegawa, Y. Kondo, J. Schneider, F. Drewnick, S. Borrmann, S. Weimer, K. Demerjian, P. Williams, K. Bower, R. Bahreini, L. Cottrell, R.J. Griffin, J. Rautiainen, J.Y. Sun, Y.M. Zhang, and D.R. Worsnop, (2007), Ubiquity and dominance of oxygenated species in organic aerosols in anthropogenically-influenced Northern Hemisphere midlatitudes, Geophysical Research Letters, 34, L13801.
[https://doi.org/10.1029/2007GL029979]